Research Methods
In this note, I want to introduce some of the famous research methods in Neuroscience. Of course, it is not possible to introduce all the different research method and it is also out of my capability to know about all of them, but based on my personal experience it would be helpful to have some understandings about at least some well known (historic) research method.
As you may know (at least as far as I observed), most of area in science does not evolve continuous/linear fashion. I think they are evolved by some big jump made by some critical findings, experiments and theories etc. In some cases those findings was not intentional (logically planned) and happened by chance and in some other cases those findings are obtained by well planned experiments
or theory by some great minds.
Regardless of whether those findings happened by chance or by plan, once those findings occurred a huge amount of subsequent research would be done around those original findings using the similar methods that are used in the original findings. So if you have some understandings about those historic findings / methods, it would be much easier to read written documents or watching lecture
video because you already know of the background context about them.
Another reason on the importance of understanding research method is as follows : Each type of Research Method produces similar types of data. For example, some method produce a data as an image (like fMRI) and some method produces the data as various different types of graphs (e.g, intra/extra cell recording, EEG etc). If you have some basic understandings (actually the more the better)
on those research method and how to interpret those results would greatly help you to understand various textbook, lectures, article, papers without putting much efforts comparing to the case where you don't have any knowledge on those research methods.
One of the biggest difficulties in Neuroscience has been around the difficulties of designing and implementing experiments. Sometimes those difficulties came from technical limitation, sometimes from lack of knowledge on what to do or sometimes from ethical reasons. I think we have difficulties from every possible reason just mentioned for human research.
Some easy way for research if everything is allowed would be to remove (or make a damage) to a certain part of nervous system and observe what happen after that. But it is rarely possible to do this kind of experiment even for higher animals not to mention human. So many of important findings about human neurosience came from the patients who got damages (lesions) by accidents or some
unknown reason.
In this section, I would introduce some of the famous / well-known patients with lesions that lead to critical findings in human neurosicience.
I came to know of Phinea Gage when I was taking a biology course about 35 years ago (sometime late 1980s) which was dealing with topics about basic brain functions. For me, it was the first time for me to hear of any patients with this kind of damage in brain.
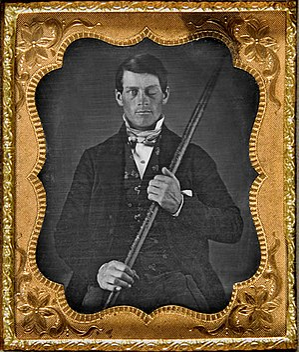
Image Source : Wikipedia
He was a railroad construction worker and got an iron bar peneterating through forehead part of his head as shown below. I was shocked by the tragedy and at the same time by the fact that he (fortunately) survived.
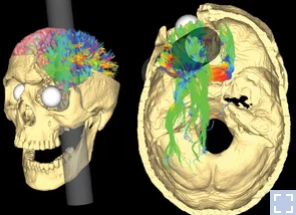
Image Source : NPR Health News
After this accident, he suffered from various personalilty issues and the observation on his behavioral changes led to the findings that specific behavior or some aspect of personality associated with a specific regions of brain. And this finding had been a trigger for a lot of subsequent researches about association between various other parts of brain and corresponding changes
in terms of behavior, emotion, cognitive processes etc.
He was an American and is widely known as H.M and may be the most famous and well-known patients. If you have been interested in neuroscience and read some of documents (books, megazines or articles in the internet) or watch lectures, I am pretty sure you might have heard of him, at least the name H.M. Since he has been mentioned in so many documents and lectures, it would be good
if you have some background information about him to follow along with those documents and lectures.
He had been a patient who greatly contributed to nuerosience, especially learning and memory process, but it was not intentional at all. He just got a brain surgery just to reduce the symptom of epilepsy, but a serious memory related problem is observed after the surgery. This unintended problem led to findings where Hipocampus plays key roles in long term memory formation and memory
may be localized (i.e, associated with a specific part of brain not associated with the all across the brain). Watch this video for the importance of findings from H.M
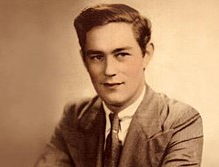
Image Source : Wikipedia
He had a bilateral medial temporal lobectomy in 1953. With this surgery, he got about two thirds of anterior hippocampus removed. The removed area were parahippocampal cortices, entorhinal cortices, piriform cortices, and amygdalae. The purpose of this surgery was to cure his epilepsy.
More detailed information of the brain lesion (damage) found from the autopsy were as follows : See this if you are interested in further details.
- the medial temporopolar cortex,
- piriform cortex,
- virtually all of the entorhinal cortex,
- most of the perirhinal cortex and subiculum,
- the amygdala (except parts of the dorsal-most nucleicentral and medial),
- anterior half of the hippocampus,
- and the dentate gyrus (posterior head and body).
- The posterior parahippocampal gyrus and medial temporal stem were partially damaged.
- Spared medial temporal lobe tissue included the dorsal-most amygdala,
- the hippocampal-amygdalotransition- area,
- ~2 cm of the tail of the hippocampus,
- a small part of perirhinal cortex,
- a small portion of medial hippocampal tissue,
- ~2 cm of posterior parahippocampal gyrus
What happened after this surgery ?
- In short, he lost the capability of form any new long term memory(this symptom is called 'Anterograde Amnesia). His skills and momery he aquired before seemed pretty intact (strictly speaking he failed to recall any memory for the 3 years of past period. this is called 'retrograde amnesia), but forming a new long term memory was impossible.
- So the doctor doing research on him had to reintroduce herself everytime they meet each other since he does not remember that he met her before.
- However his procedural memory formation (e.g, reading words in mirror(i.e, backward reading)) seems intact (@45:00 this lecture)
I was not able to find YouTube video showing his symptom directly (he passed away before sharing video over internet is so common), but I found some videos explaining about him like this, this
Clive Wearing is a British former musicologist, conductor, tenor and keyboardist. He had similar brain lesion as H.M (i.e, lesion in Hipocampus) but he got this from his illness not by any physical surgery or accidents. He lost the functionality of large portions of Hippocampus and temporal lobe which plays crtical
function in memory process.
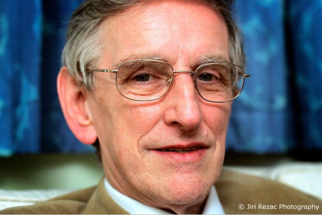
Image Source : Alchetron
After the illness, he has been suffering serious Amnesial.
- He lost most of his his past episodic memory(retrograde amnesia) and is not able to form new long term memory (anterograde amnesia).
- He had around 20 years period of retrograde amnesia meaning his memory about past 20 years has gone (@53:40 this lecture).
- His new memory goes only around 20~30 seconds and then reset. He is like waking up fresh every 20~30 seconds.
- His non-declaritive memory (e.g, procedural memory like playing piano) was intact.
Personally Clive Wearing is more familiar to me than H.M because I got several YouTubes (watch this , this) showing / describing details of the problem he is suffering, whereas I was not able to find any video about H.M showing the live
behavior of H.M.
The nervous system, whether in a human, a mouse, or even a fruit fly and squid, is a symphony of electrical impulses, a complex orchestra of neurons firing in harmony to create thoughts, behaviors, and physiological responses. Electrophysiological recording serves as our conductor's baton, allowing us to eavesdrop on this intricate ballet of electrical activity across diverse species and scales.
From the precise measurements of individual neurons within a petri dish or within a specific body part to the sweeping brainwaves captured by electroencephalography (EEG) in freely moving animals, electrophysiological techniques offer an unparalleled window into the inner workings of the nervous system.
In this techblog series, we will embark on a comprehensive exploration of electrophysiological recordings, showcasing their versatility and far-reaching applications in both basic research and clinical settings. We'll delve into the diverse methods used to capture these electrical signals, from intracellular and extracellular recordings in isolated cells to multi-electrode arrays (MEAs) that monitor the activity of entire neural networks.
I think the origin of the single cell measurement in electrical way would be the test with a super big nerve cell (neurson) from a squid shown below. Especially thanks to this, we could learn such a detailed mechanism of Action Potential. As shown below, the size of a nerve cell in this squid is so huge that you can see the single nerve cell with naked eyes and
put an electrode directly inside of the cell with the technology decades ago. This technique measuring the electric potential (voltage) of inside of single neuron called Voltage Clamp / Patch Clamp technique.
With the continuous evolution since this research, both electronics and equipment manufacturing technology has been developed drastically, we would do the same type of measurement for higher animals which has much thiner / smaller nerve cells (If you are seriously interested in the intra cell recording with modern technology, I would recommend this article (Whole-Cell Recording of Neuronal Membrane Potential during Behavior).
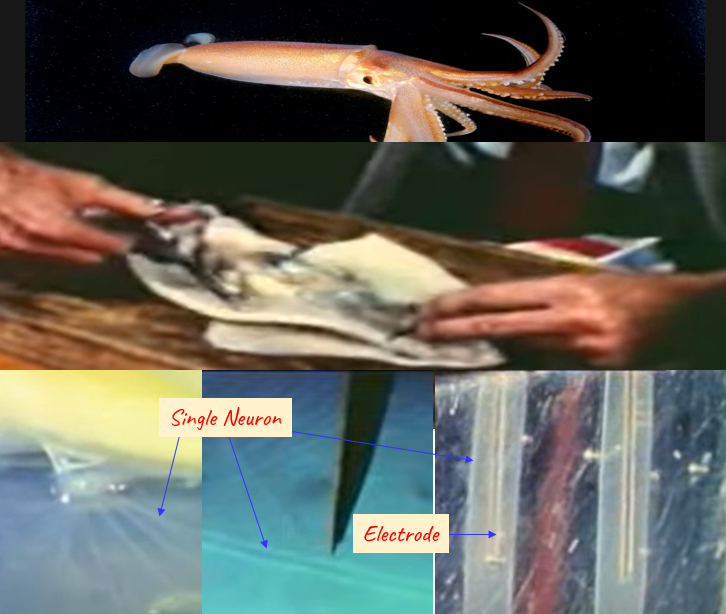
Source : YouTube
Achievement/Application
-
Discovery of Action Potentials : This method enabled precise measurements of electrical signals inside a single neuron, leading to the groundbreaking Hodgkin-Huxley model (1952), which explained how action potentials are initiated and propagated in neurons.
-
Ion Channel Characterization : Voltage clamp allowed researchers to isolate and study specific ion channels (e.g., sodium, potassium, calcium), revealing their roles in neuronal excitability, signaling, and neurotransmitter release.
-
Drug Development & Pharmacology : Pharmaceutical studies use patch-clamp to test how drugs affect ion channels. It's crucial in developing treatments for epilepsy, cardiac arrhythmia, and pain.
-
Synaptic Physiology : Enabled the study of postsynaptic potentials and helped unravel mechanisms of synaptic plasticity such as long-term potentiation (LTP), critical for learning and memory.
-
Brain Slice & In Vivo Applications : Advanced forms like whole-cell patch clamp can be applied in brain slices or even in vivo in live animals, giving insights into how neurons behave during natural behaviors.
-
Development of Computational Models : Intracellular recordings provide the data needed for biophysically accurate neuron models, which are used in AI and neural simulation platforms (e.g., NEURON, Brian).
Challenges/Limitation
-
Technical Difficulty : Inserting a microelectrode into a single neuron without damaging it requires high skill and precision. Even more challenging for smaller mammalian neurons than giant squid axons.
-
Limited to One or Few Cells : Typically allows recording from only one or a few cells at a time, making it hard to study large-scale network activity or interactions among many neurons.
-
Cell Damage & Stability : The electrode insertion can disrupt the cell membrane or cause cell death. Recordings are often short-lived due to this stress.
-
Invasiveness : Especially in in vivo setups, the technique is highly invasive and may alter the natural state of the tissue or neuron.
-
Accessibility : Not all brain regions or neuron types are easily accessible, particularly deep or densely packed structures.
-
Data Complexity & Interpretation : The data requires complex analysis to infer channel dynamics, membrane properties, and synaptic input, often needing computational support.
Extra Cell Recording is also measuring the electrical activity of neurons like intro cell recording. Extra cellular recording measures the electrical activities outside of nerve cells (i.e, the measurement elelctrode is placed outside of the cell) whereas the measurement electrode is placed inside of the cell in Intra cell recording. Since the measurement electrode placed outside
of a cell, it is not guaranteed that the measured activity is from a single cell. It can be the measurement of a single cell or multiple cells around the electrode.
Advantages of Extra Cell Recording comparing to Intra Cell Recording would be that it does not require such a sophisticated setup as in intra cellular recording, meaning that it is much easier to set up the test.
Achievement/Application
- Multi-Neuron Monitoring : Can record many neurons simultaneously, making it ideal for population-level or network-level activity studies, especially in behaving animals.
- Behavioral and Systems Neuroscience : Widely used in awake, freely moving animals, making it ideal for correlating neural activity with sensory stimuli, decisions, and movements.
- Brain-Machine Interfaces (BMI) : Extracellular spikes form the basis for neuroprosthetics and BCI (brain-computer interface) research (e.g., controlling robotic arms via cortical signals).
- Neural Coding Studies : Allows investigation into how groups of neurons encode sensory inputs or behaviors through spike timing and rate.
- Clinical Diagnostics : Basis for electrocorticography (ECoG) and electroencephalography (EEG) in humans, essential in epilepsy, sleep studies, and brain health monitoring.
Challenges/Limitation
- Ambiguity of Signal Source : Difficult to know which specific neuron generated a recorded spike. Signals often represent multiple nearby neurons.
- Signal-to-Noise Ratio (SNR) : Since it's measured outside the cell, extracellular signals are weaker and more susceptible to noise.
- Lack of Subthreshold Information : Cannot capture subthreshold events (e.g., synaptic potentials, membrane resistance changes), limiting insight into intracellular dynamics.
- Spike Sorting Complexity : Requires complex computational algorithms to separate overlapping spike waveforms from different neurons.
Micro Electrode Array(MEA) is a special type of electrode (bar or thread like electrode) in which has multiple electrodes embedded as illustraded below. I think the biggest advantage of MEA is that it can record the neuron activities of multiple layers of a cortex region simultaneously as shown below.
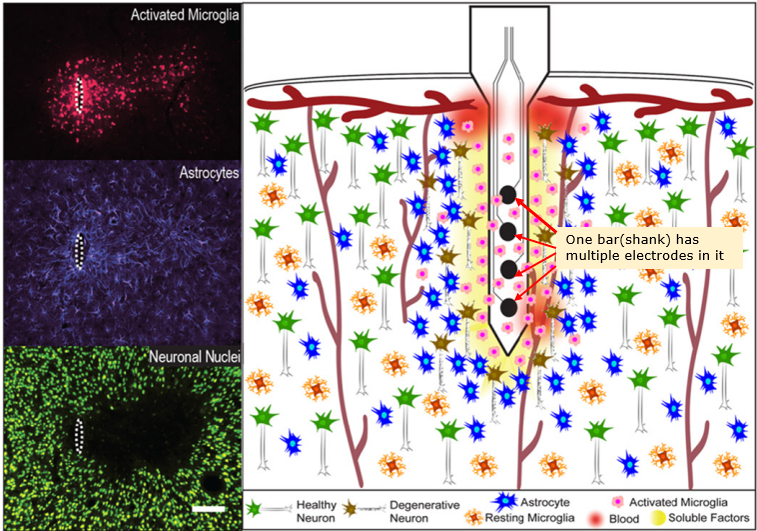
Image Source : Progress Towards Biocompatible Intracortical Microelectrodes for Neural Interfacing Applications
One of the most common / famous type of MEA would be Utah Electrode Array (UEA). It is made up of basic components called shank(a bar like structure). The shank is a bar like silicon wafer on which multiple electrodes are deposited as shown below.
It was developed at the University of Utah in the late 1970s and early 1980s. It was developed as part of a research project aimed at developing a brain-machine interface that could be used to control prosthetic devices, such as a robotic arm or a computer cursor, using the activity of neurons in the brain. The UEA has since been widely used in research and clinical settings, and has been instrumental in advancing our understanding of the brain and the development of treatments for neurological
disorders.
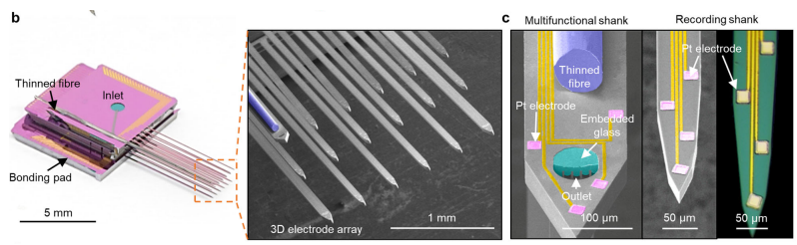
Image Source : 3D high-density microelectrode array with optical stimulation and drug delivery for investigating neural circuit dynamics
If you make a linear array(a vector) of shanks, you can record the neural activities of mutiple layers across a certain span of the cortex simultaneously.
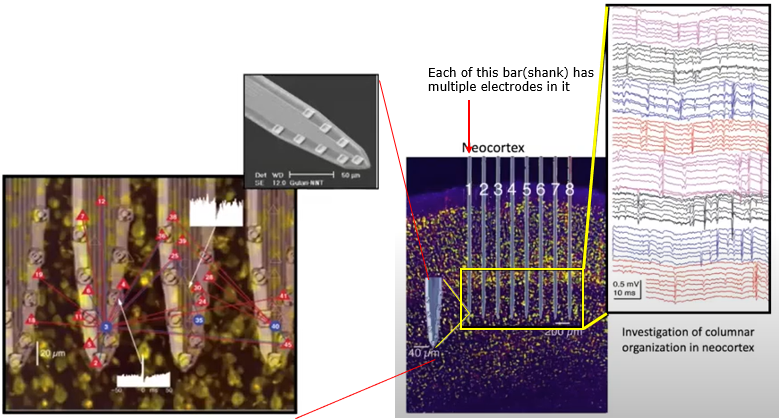
Image Source : Electrode Technology with Dr. Rio Vetter | Webinar
If you make a 2D array(a matrix) of shanks, you can record the neural activities of mutiple layers across a certain area of the cortex simultaneously.
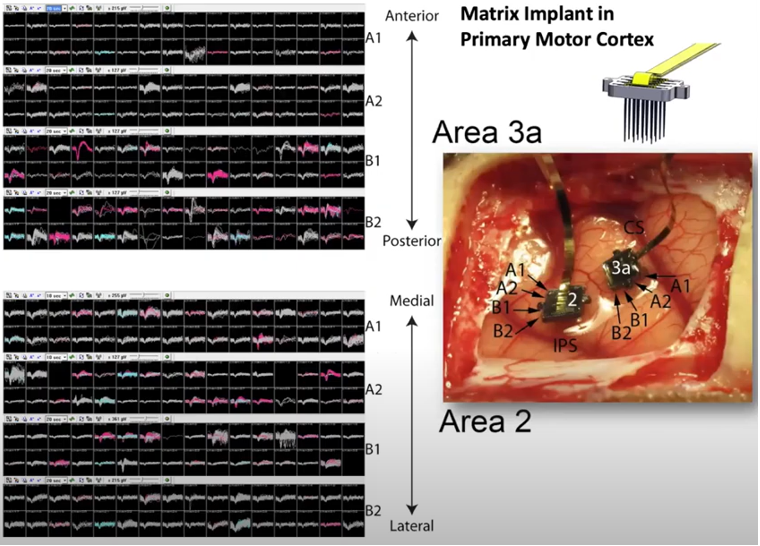
Image Source : Electrode Technology with Dr. Rio Vetter | Webinar
I think the most recent technology of MEA (as of writhing this note : Jan 2023) would be MEA being used in Neuralink. This electrode is a flexible structure in which a linear array of electrodes are embedded. Eac of the thread can be planted into the cortex of the brain by a special machine (a medical robot).
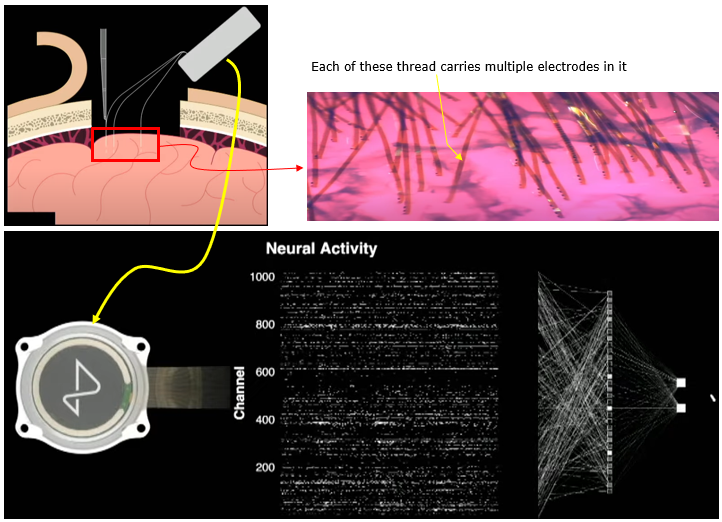
Image Source : Neuralink Show and Tell, Fall 2022
Achievement/Application
-
Simultaneous Multi-Layer Recording : MEAs (especially Utah Arrays and modern 3D arrays) can record from multiple neurons across different cortical layers or regions simultaneously, offering a 3D view of brain activity.
-
High-Density, High-Channel Count : Advanced versions (e.g., Neuralink threads) support hundreds to thousands of channels, allowing fine-grained mapping of neural circuits and more powerful decoding.
-
Prosthetics and Brain-Machine Interfaces (BMI) : Used in systems to control robotic arms, cursors, or speech synthesis, especially by decoding motor or sensory intentions (e.g., Utah Array in primary motor cortex).
-
Chronic Implantation : Devices like the Neuralink N1 are designed for long-term implantation, enabling longitudinal tracking of neural changes and real-time control in clinical applications.
-
Spatial & Functional Mapping : 2D/3D arrays allow researchers to map functional architecture (e.g., orientation columns, motor maps) and even study disease progression (e.g., neurodegeneration, epilepsy).
-
Multimodal Capability : Some modern MEAs integrate optogenetics, chemical delivery, and optical fibers to modulate and monitor brain activity concurrently.
-
Tool for Fundamental Neuroscience : MEAs provide detailed spike timing and activity data to study synaptic connectivity, population coding, and neural dynamics.
Challenges/Limitation
-
Tissue Damage & Immune Response : Insertion of MEAs causes mechanical damage and chronic immune responses (e.g., microglial activation, astrocytic scarring), as shown in histological images.
-
Signal Degradation Over Time : Chronic implants may suffer from signal loss or drift, especially in rigid structures like the Utah array, due to gliosis and electrode corrosion.
-
Surgical Complexity : Requires invasive craniotomy and precise implantation; in advanced systems like Neuralink, robotic assistance is needed to insert flexible threads.
-
Material Biocompatibility : Must balance flexibility and stiffness—too stiff damages tissue, too soft fails to penetrate or loses signal quality.
-
Power & Data Bandwidth : High-channel count systems (e.g., >1024 channels) produce massive data, challenging for real-time processing, compression, and wireless transfer.
-
Localization and Specificity : While they provide broad coverage, pinpointing exact synaptic or subthreshold events like in intracellular recording is not possible.
EEG stands for ElectroEncephaloGram. This is non-invasive technique to measure the activity of brain. As shown in the picture below, it does not require the opening of the brain... just put a lot of measurement electrode on the skull. Obvious it is much easier to setup and perform the measurement comparing to cellular recording, but it is not possible to associate the measured brain
activity to a specfic nerve cells.
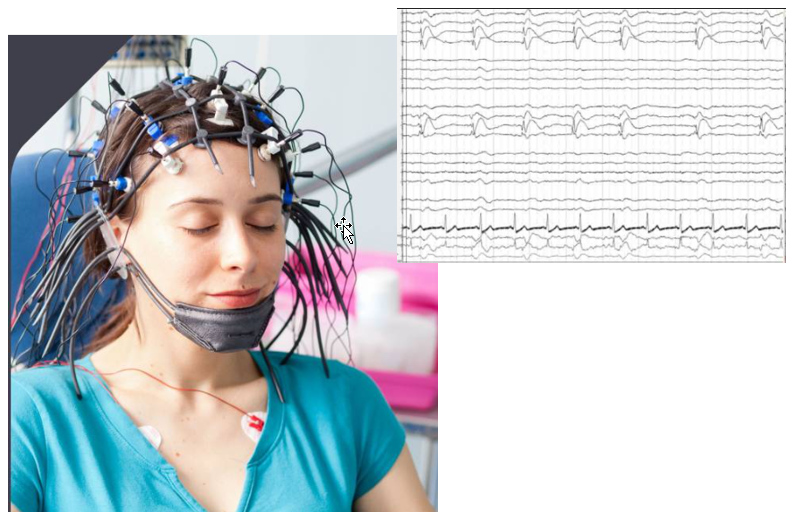
Achievement/Application
-
Non-Invasive Brain Monitoring : EEG is one of the few techniques that can measure brain activity without surgery, making it safe, repeatable, and ideal for clinical and research use.
-
High Temporal Resolution : Excellent for capturing rapid neural dynamics—on the order of milliseconds, suitable for analyzing event-related potentials (ERPs), brain rhythms, and seizure activity.
-
Clinical Diagnostics : A cornerstone in diagnosing epilepsy, sleep disorders, brain death, and head injuries. Frequently used in ICUs, sleep labs, and neurology clinics.
-
Cognitive Neuroscience Research : Used to study attention, language processing, decision-making, emotion, and perception. ERP components (e.g., P300, N170) are widely analyzed.
-
Brain-Computer Interfaces (BCIs) : Enables real-time control of devices using neural activity, particularly in patients with disabilities (e.g., controlling a cursor or wheelchair).
-
Portable and Affordable : Compared to fMRI or invasive recording methods, EEG equipment is relatively low-cost, easy to transport, and usable in diverse settings—including field research.
Challenges/Limitation
-
Low Spatial Resolution : EEG measures summed electrical activity from the scalp, resulting in poor localization. It cannot pinpoint activity to specific neurons or cortical layers.
-
Signal Distortion by Skull and Scalp : Electrical signals are attenuated and smeared by the skull, reducing signal clarity and introducing mixing from neighboring brain regions.
-
Sensitivity to Artifacts : Prone to noise from muscle movements, eye blinks, heartbeat, and external electrical sources, which must be carefully filtered.
-
Inverse Problem : Even with advanced modeling, the task of deducing where in the brain a specific signal originated from the scalp data is mathematically ill-posed and underdetermined.
-
No Subthreshold or Synaptic Info : EEG reflects population-level postsynaptic potentials but cannot resolve synaptic integration, ion channel dynamics, or action potentials of single neurons.
-
Limited to Cortical Surface Activity : EEG primarily captures signals from the outer cortical regions—activity in deeper brain structures (e.g., hippocampus, thalamus) is barely or not at all detectable.
'f' in fMRI means 'Functional' MRI. This technology was developed early 1990 and have been one of the most important contributors in brain science even until now.
This equipment(technology) can take picture of brain image highlighting the specific area of the brain which are 'functioning' (called 'Active') at the time of the shot. Usually it indicates the active region as redish color (the more redish the more active) and indicates the less active area in bluish or other darker colors as shown below. Thanks to this techniq, scientist has been able
to figure out which part of the brain is associated with what kind of mental activity without relying on patients with specific lesions or physical operation etc.
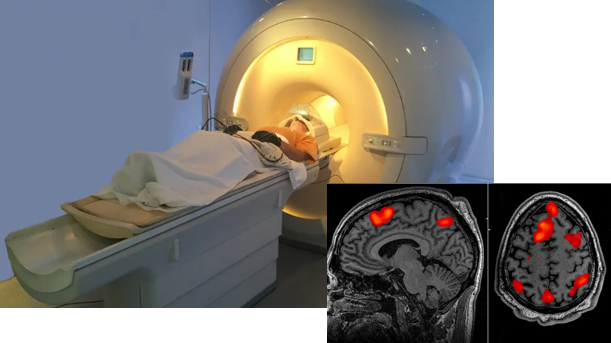
Image Source : PsychCentral
Even though the technology is complicated, the logic of the research is pretty straight forward. Let the persion in the equipment perform a certain mental activity and see which part of the brain become active at the time of the activity.
For example, it goes like this
- i) Show the person a picture
- ii) Observe which part of the brain gets activated (an example shown below. You would recognize the occiptal lob is highly activated)
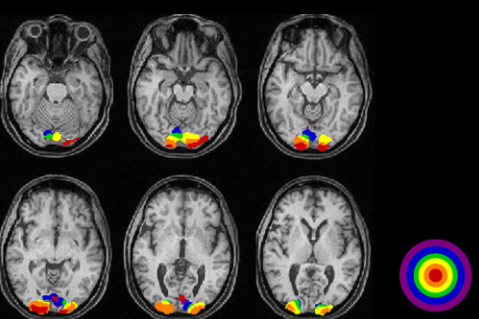
Image Source : VISUALLY EVOKED POTENTIALS BY DONNELL J. CREEL - WEBVISION
NOTE : If you want to know of more technical details of this technique, I think this article would be very good starter and then you check further on other articles and videos in the YouTube and
Reference section.
Achievement/Application
- Non-Invasive Mapping of Brain Function : fMRI allows researchers to identify which brain regions are active during specific tasks (e.g., viewing images, solving problems, feeling emotions) without surgery or lesions.
- Whole-Brain Coverage : Provides data from the entire brain (including deep structures like the amygdala, hippocampus, and thalamus), unlike EEG or MEA which are biased toward surface or implanted regions.
- Localization of Cognitive Functions : Helped map brain functions such as:
- Vision (occipital lobe)
- Language (Broca’s/Wernicke’s areas)
- Motor control (motor cortex)
- Emotion (limbic system)
- Decision-making (prefrontal cortex)
- Clinical Pre-Surgical Planning : fMRI is used before brain surgery (e.g., for tumor removal) to avoid damaging critical functional areas (like language or movement zones).
- Resting-State fMRI (rs-fMRI) : Reveals intrinsic functional connectivity networks (like the Default Mode Network) while subjects are at rest—vital in disorders like depression, Alzheimer's, autism.
- Psychiatric and Developmental Research : fMRI has been widely applied in studying
- Schizophrenia, bipolar disorder, ADHD, autism
- Neurodevelopment and aging
- Effects of meditation, drugs, therapy, etc.
Challenges/Limitation
- Low Temporal Resolution : fMRI measures changes in blood flow (BOLD signal), not electrical activity. This signal is delayed by several seconds, limiting precision in fast neural dynamics.
- Indirect Measure of Neural Activity : The BOLD signal reflects oxygenation changes, which are only correlated with neural activity—making it an indirect and complex proxy for true firing rates.
- Expensive and Complex : Requires a large, shielded facility and highly trained personnel, making it expensive and less accessible than EEG.
- Motion Sensitivity : Subject must remain very still—even small movements can corrupt data. Hard to use with young children, animals, or in naturalistic behavior.
- Spatial Resolution Limited by Hemodynamics : Although fMRI has millimeter-scale spatial resolution, it cannot resolve individual neurons or very fine cortical layers.
- Susceptibility to Artifacts : Affected by physiological noise (heartbeat, breathing) and scanner drift, requiring careful preprocessing.
- Ethical Concerns in Interpretation : High risk of overinterpretation of "activation maps" in popular media. Also, individual differences in brain anatomy/function can complicate analysis.
DTI stands for Diffusion Tensor Imaging. Technically the term 'Diffusion Tensor' itself explains a lot but for most people like me, it doesn't make much sense. For for the layman like me in this area, you may just think of this as a technology that can measure the activity of water molecules mostly along with axon of neurson. Since the axon is the part of the neuron connecting a part
of nerve system to another part of the system, this image can visualize the complex pathways in nerves system (e.g, in brain) as shown below.
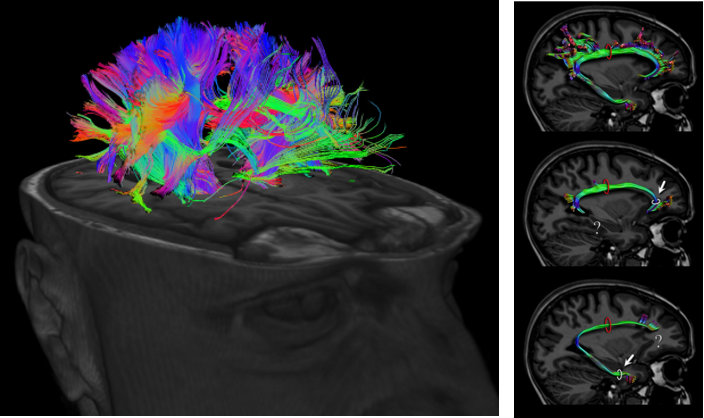
Image Source : Imagilys and eBrain
NOTE : If you want to know of more technical details of this technique, I think this article (Diffusion MRI fiber tractography of the brain) would be the best explaining article
about DTI.
Achievement/Application
- Visualization of White Matter Tracts : DTI allows non-invasive mapping of axonal fiber bundles, which are the communication highways of the brain, such as the corpus callosum, arcuate fasciculus, and corticospinal tract.
- Understanding Brain Connectivity : DTI is the foundation of connectomics—mapping how different brain regions are structurally connected (the "wiring diagram" of the brain).
- Surgical Planning : Helps neurosurgeons avoid damaging critical white matter tracts during tumor removal or epilepsy surgery by mapping nearby fiber pathways.
- Neurological & Psychiatric Research : Widely used to study multiple sclerosis, stroke, traumatic brain injury (TBI), Alzheimer’s, autism, and schizophrenia, where white matter integrity is affected.
- Developmental and Aging Studies : Tracks changes in white matter during childhood development or age-related decline, revealing how connectivity patterns evolve.
- Early Biomarker Discovery : Alterations in fractional anisotropy (FA)—a DTI metric—may serve as early indicators of disease before structural damage appears on traditional MRI.
- Structural-Functional Integration : DTI is often combined with fMRI to correlate functional activation with structural pathways, supporting network-level understanding.
Challenges/Limitation
- Limited to White Matter : DTI only tracks anisotropic diffusion, which occurs mainly along myelinated axons—it doesn’t reveal functional activity or gray matter dynamics.
- Indirect Measurement : It doesn’t image axons directly, but rather the movement of water molecules that are constrained by axonal geometry. This means it infers structure, not measures it directly.
- Vulnerability to Artifacts : Susceptible to motion, eddy currents, and partial volume effects, which can distort fiber tracking accuracy.
- Crossing Fibers Ambiguity : In voxels where multiple fiber tracts cross or kiss, standard DTI cannot resolve the direction clearly—more advanced models (e.g., HARDI, CSD) are needed.
- No Temporal Information : DTI provides static, structural images and does not capture dynamic changes over time. Unlike EEG or fMRI, which measure brain activity as it unfolds (electrical signals or blood flow changes over seconds or milliseconds), DTI captures a snapshot of how water molecules diffuse in the brain at the time of scanning. It shows how regions are anatomically connected (e.g., fiber tracts) but tells us nothing about when or whether those regions are active during a task or rest.
- Complex Interpretation : Metrics like FA, MD, AD, and RD require careful interpretation, and variations can arise from factors unrelated to pathology (e.g., hydration, myelination, fiber density).
Transcranial Magnetic Stimulation is a technique that can generate a strong magentic field at a spefici location in the brain without any surgery (i.e, it is non-invasive technique). It would be the biggest advantage of TMS that the technology can stimulate a specific part of the brain without surgery.
As you know neruon activity is performed by electric current changes. This electric current changes would induce magnetic field. This magentic field can be interfered/interfacted by the magnetic field applied by TMS. This is a short story on how TMS works.
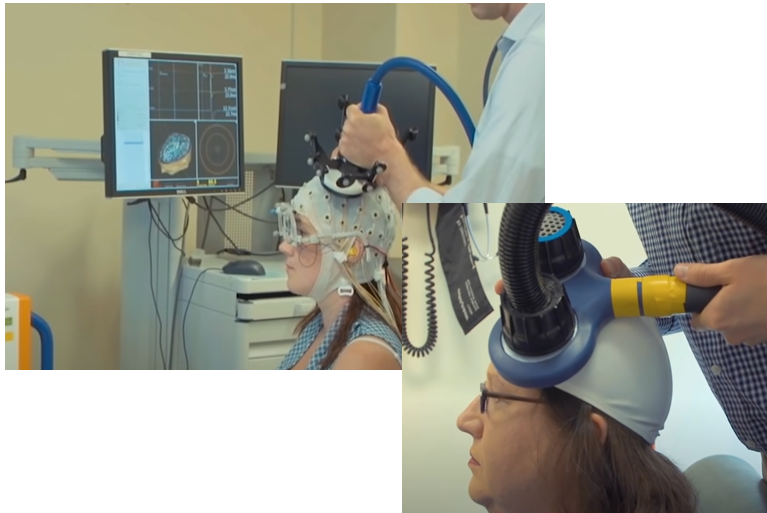
Achievement/Application
-
Non-Invasive Brain Stimulation : TMS allows stimulation of specific brain regions without surgery, making it much safer and more accessible compared to deep brain stimulation or implantable electrodes.
-
Therapeutic Use in Depression : One of the most FDA-approved clinical uses is for treating major depressive disorder (MDD), especially in drug-resistant cases. Repeated TMS (rTMS) can significantly improve mood and cognitive function.
-
Probing Causal Brain Function : Unlike passive techniques (e.g., fMRI, EEG), TMS can perturb brain regions, allowing researchers to infer causal relationships between brain areas and behavior.
-
Functional Brain Mapping : TMS is used in research to map cortical excitability, for example identifying motor cortex regions before neurosurgery or investigating the role of specific areas in memory, attention, or speech.
-
Combination with EEG or fMRI : Concurrent TMS-EEG or TMS-fMRI setups allow researchers to observe how stimulation propagates through networks and influences oscillatory brain activity.
-
Treatment for Neurological Disorders : Investigated for stroke recovery, chronic pain, OCD, migraine, PTSD, Parkinson’s disease, and more.
Challenges/Limitation
-
Limited Depth Penetration : TMS can only reliably stimulate superficial cortical areas (e.g., prefrontal cortex, motor cortex). Deep brain regions (e.g., hippocampus) are largely inaccessible.
-
Spatial Resolution : The stimulated area is about 1–2 cm wide, which is relatively coarse. Targeting subregions within the cortex can be imprecise without neuronavigation.
-
Inter-Individual Variability : The effects of TMS can vary across individuals due to skull thickness, cortical folding, and baseline brain state, requiring personalized targeting.
-
Temporary Effects : Behavioral or therapeutic effects of single-pulse or short TMS sessions are transient. Long-term effects often require daily sessions over weeks.
-
Safety Risks : While generally safe, high-frequency or poorly monitored TMS may cause side effects like:
-
Scalp discomfort
-
Muscle twitching
-
In rare cases, seizures
-
No Direct Recording Capability : Unlike MEA or EEG, TMS modulates brain activity but does not record it—hence often combined with other tools to interpret effects.
By far the best technology that shows the most detailed structure of cellular structure would be the electron microscope. That is, electron microscope is the technology that has be the best magnifying power. But a problem of this technique is that it requires a lot of effort to make preparation for the observation. It would be the best if CT or MRI has such a resolution as electron microsoft,
but unfortunately it is only in our wish list.
One of the best alternative would be to use electron microscope like CT. In short, it takes out small volume of brain tissue and slices it into a lot of layers. Each of the layers are phographed under the electron microscope. If you color a specific neurons apeared on every slices and reconstruct a 3D image, you can get some images as shown below.
If you are interested in the further details of this technology, I think this is the best introductary video : Connections in a Cube/ Cell, July 30, 2015 (Vol. 162, Issue 3) and How brains see
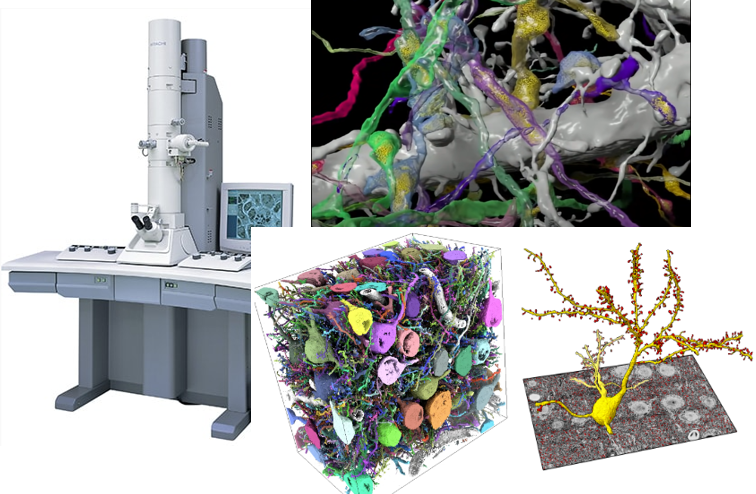
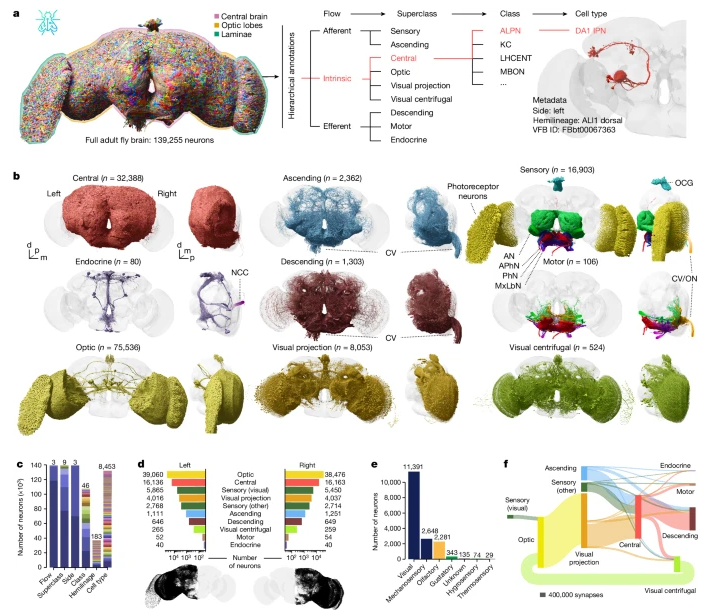
Image Source : Whole-brain annotation and multi-connectome cell typing of Drosophila
Achievement/Application
-
Unmatched Spatial Resolution : vEM offers nanometer-scale resolution, revealing the ultrastructure of neurons, including:
-
Detailed Connectome Mapping : Enables reconstruction of complete neuronal circuits (axon-to-dendrite connections) in 3D. The landmark "mouse retina connectome" and "Drosophila brain map" were built using this.
-
Precise Structural Ground Truth : Serves as the gold standard for verifying results from lower-resolution techniques like light microscopy, calcium imaging, or fMRI.
-
Revealing Micro-Architectural Patterns : Helps uncover:
-
Synaptic density and diversity
-
Glial-neuron relationships
-
Local circuit motifs (feedforward, feedback loops)
-
Data for AI & Simulation Models : Provides realistic anatomical models used in brain simulations (e.g., Blue Brain Project) or in training AI-based segmentation tools.
-
Cell Type Classification : With detailed structure and location, vEM supports morphological classification of neurons beyond just functional labels.
Challenges/Limitation
-
Extremely Data-Intensive : A single cubic millimeter of brain tissue can yield petabytes of data, requiring immense storage, computation, and AI-based segmentation.
-
Destructive & Non-Live Imaging : Requires physical slicing and sample preparation, which destroys the tissue. No time dynamics or live observation is possible.
-
Preparation Complexity : Tissue needs to be:
-
Chemically fixed
-
Stained with heavy metals (e.g., osmium)
-
Dehydrated and embedded in resin
-
Thin-sectioned (~40–70 nm per slice)
-
Labor-Intensive Manual Annotation : While AI helps, many neural reconstructions still require manual proofreading, especially for dense or tangled circuits.
-
Limited Volume Coverage : While volume electron microscopy has achieved stunning results — including the complete mapping of the fruit fly brain — it remains technically and computationally prohibitive for larger brains, such as those of mammals. For instance, imaging a mouse brain or human cortical region at nanometer resolution would require massive data storage, years of imaging, and significant manual or AI-based segmentation. Thus, whole-brain mapping using vEM is currently feasible only for small brains or localized regions in larger animals.
-
No Functional Information : Captures structure, but cannot measure activity, neurotransmitter levels, or plasticity. Often combined with physiology for functional insight.
CLARITY/Tissue Clearing
I think this is very intriguing technique to me. In terms of resolution, I think Electron microscopy shown above would be the best but it would be extremely difficult to visualize the scale of whole brain (even for the small brain like rat brain). This technique is designed to make the whole brain (or large chunks of brain tissue) transparent so that we can look insde of the brain without
cutting it open or slicing it out. The technique originally named as CLARITY and then with variations of the technique the types of techniques that can make the tissues/organ transparent called 'Tissue Clearing Technique'.
First let's think of why most of tissue / organ (like brain) is not transparent as shown in [A] and we cannot see through it without cutting it open. It is mainly because of lipid layer of cell membrane. So the idea of making the tissue/organ transparent is to wash out the lipid. But if you just wash out the lipid layer, it will break all the cells and internal structure of the cell.
It is not making it transparent, it is making a porridge :). The smart trick in this technology of making them transparent is to form gelish mesh through the whole organ/tissue and then wash out the lipid layer. Then the mesh will keep all the non-lipid biomolecules (e.g, protain) as it is located before as shown in [B].
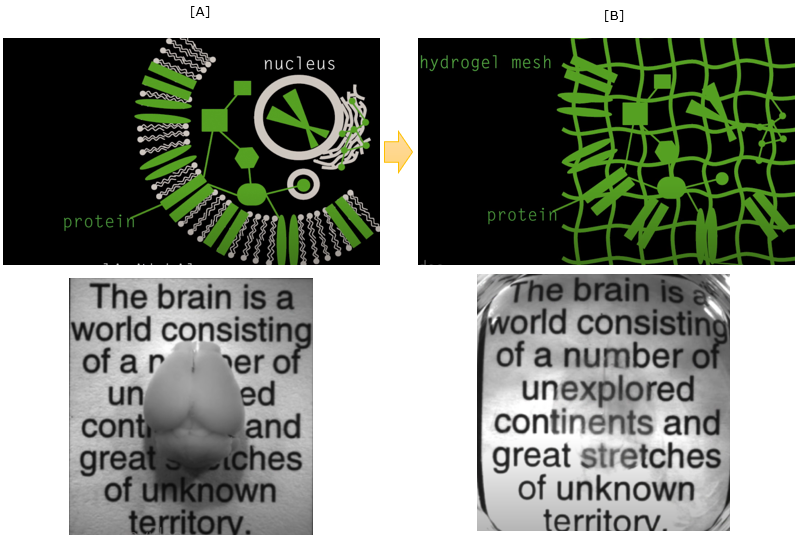
Image Source : See-through brains
Even though the technology is fancy, just making the tissue transparenet would not do much. To get more meaningful data out of the transparent tissue, you would need various additional techniques like staining and microscopy technologies. If you combine all those technologies properly, you woult get such a fancy and detailed images as shown below.
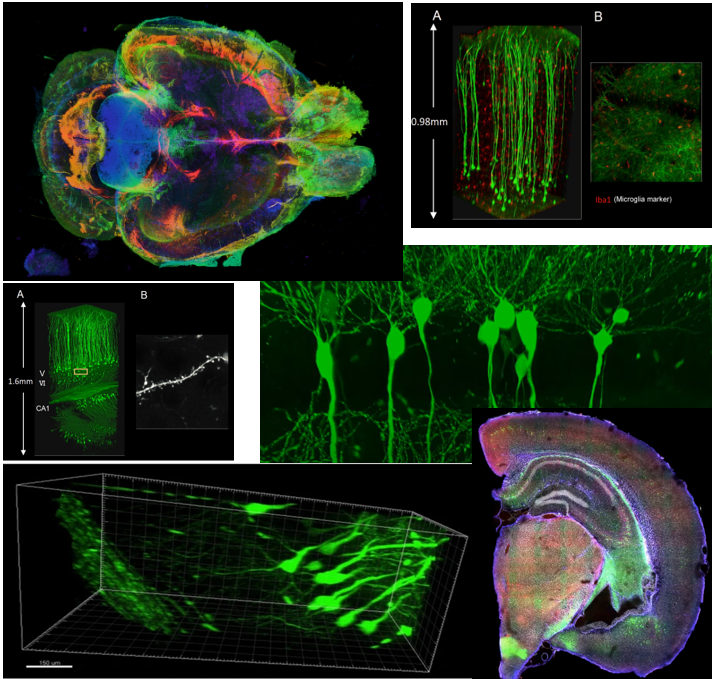
Image Source : Compiled from various documents listed in Reference
Achievement/Application
-
3D Visualization of Intact Brain Tissue : CLARITY and similar clearing methods (e.g., iDISCO, uDISCO, CUBIC) make tissues optically transparent, allowing deep 3D imaging of entire brains or organs while preserving structural and molecular content.
-
Preservation of Biomolecular Structures : Lipids are removed while proteins, nucleic acids, and structures are preserved via hydrogel embedding, enabling detailed imaging without disrupting the architecture.
-
Whole-Brain Connectomics : When combined with light-sheet microscopy and staining (e.g., for cell types, synapses, or activity markers), researchers can trace long-range neuronal projections across the entire brain.
-
Compatibility with Fluorescent Labels : Works well with genetically encoded markers (e.g., GFP), immunolabeling, or chemical stains—ideal for mapping specific neuron types, glial cells, or molecular activity markers.
-
Phenotyping in Disease Models : Used to study neurodegeneration, cancer, stroke, and psychiatric disorders by revealing pathological changes across the whole brain in animal models.
-
Human Brain Tissue Imaging : While slower and more complex, CLARITY has been adapted to postmortem human brain samples, enabling rare 3D reconstructions of human cortical and subcortical regions.
Challenges/Limitation
-
Long Processing Time : Clearing and staining large tissues (e.g., whole mouse brain) can take days to weeks, depending on the method and tissue size.
-
Imaging Infrastructure : Requires specialized microscopes, like light-sheet, confocal, or two-photon setups, with high working distance and refractive index matching.
-
Signal Attenuation : Despite clearing, light scattering and signal decay can still occur in deeper layers, especially if staining is weak or uneven.
-
Not Quantitatively Perfect : While 3D views are stunning, quantitative analysis (e.g., absolute protein levels or signal intensity) can be skewed due to diffusion limits of antibodies or clearing inhomogeneity.
-
Tissue Expansion or Shrinkage : Some methods cause distortions—expansion or shrinkage—during hydrogel embedding or solvent clearing, affecting anatomical accuracy.
-
Resolution Depends on Imaging Method : The technique itself is not high-resolution; it relies on downstream imaging, meaning that the final detail depends on microscope quality and labeling strategies.
I think one of the most important technology in neuroscience (actually not only in neuroscience, but also biology in general) would be staining (dying) technology. Like most of other cells in our body, nerve cell (neuron) would just look transparent or translucent when you just observe it under microscope. It is the staining technology that make those cells look clear and outstanding.
Golgi staining would be the oldest and most widely used staining technology for neuron observation. Just a few examples shown below would tell you what it is like.
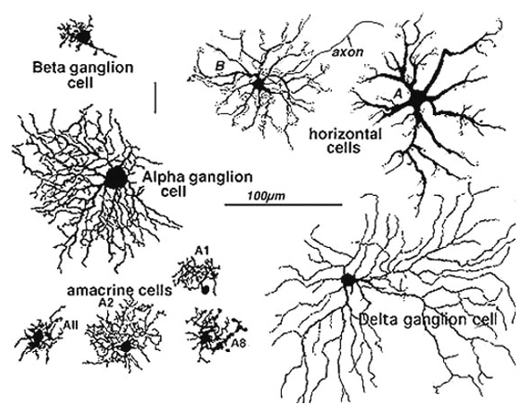
Image Source : Webvision: The Organization of the Retina and Visual System
Achievement/Application
-
First Technique to Visualize Individual Neurons : Invented by Camillo Golgi in the late 19th century and later used by Santiago Ramón y Cajal to reveal the structure of neurons, forming the foundation of modern neuroscience.
-
Revealed the Neuron Doctrine : Made it possible to visualize individual neurons in their entirety, supporting Cajal's revolutionary view that the brain is composed of discrete cells, not a continuous reticulum.
-
Morphological Classification : Enabled scientists to classify neurons (e.g., pyramidal cells, Purkinje cells, ganglion cells) based on shape, size, and dendritic arborization.
-
Still Used for Architectural Mapping : Despite its age, Golgi staining is still used for morphological studies and dendritic spine analysis, especially in developmental, aging, and disease research.
-
Visualization of Rare Neurons : Randomly stains a small percentage of neurons—ironically, this is an advantage, as it avoids visual clutter and allows clean tracing of individual cells.
-
Foundation for Modern Cell Labeling Techniques : Inspired development of more targeted and specific cell-labeling methods (e.g., immunostaining, genetic labeling, Brainbow).
Challenges/Limitation
-
Random Labeling : Golgi staining is non-selective and random, meaning you cannot control which neurons are stained—no targeting by type or function.
-
Low Throughput : Visualization and analysis are typically manual and time-consuming, making it hard to use in high-volume or large-scale datasets.
-
No Molecular Information : Golgi stain reveals morphology but not protein expression, neurotransmitter type, or synaptic markers—unlike immunostaining.
-
Static and Postmortem : Applicable only to fixed tissue; does not provide any functional or live information.
-
Staining Inconsistency : The chemical process can be finicky, with variable results in staining quality, background noise, and tissue penetration.
-
Not 3D-Friendly by Itself : Golgi staining is done on thin tissue sections; full 3D reconstructions are possible but require extensive manual tracing and stacking.
Simply put, Fluorescent staining is a technology to let a special fluorescent chemicals (called fluorescent probe) stick to specific intracellular structures (e.g, protein, neucleotides) and observe it with a specially designed microscope. There are several different ways to let fluoresent chemicals stick to nerve cells. Some of the typical ways are :
- Soaking the whole speciman in a solution containing the fluorescent probe
- Injecting the fluoroscent probe directly into the neuron (the micro tubes being used for intracellular recording is often used to inject the probe into the cell).
- Using immunoglobins tied up with fluoroscent probes.
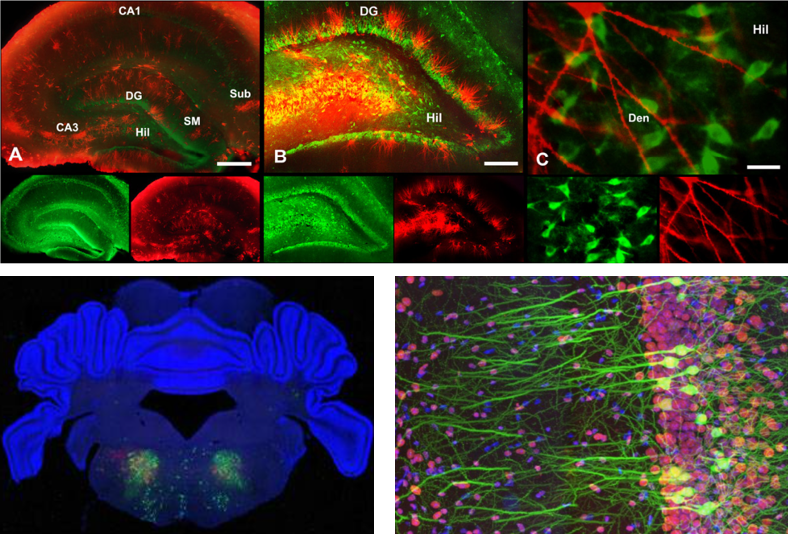
Image source : here, here and here
Achievement/Application
-
Cell-Type Specific Labeling : Fluorescent stains can be tailored to highlight specific cell types, organelles, proteins, nucleic acids, or synapses—enabling deep analysis of cellular function and organization.
-
High-Contrast Imaging : Fluorescent probes provide exceptionally clear and high-resolution images under confocal, epifluorescence, or two-photon microscopy, making even fine structures like dendritic spines visible.
-
Multichannel Imaging (Color Coding) : Using different fluorophores, multiple targets can be labeled simultaneously, allowing for:
-
Structural mapping
-
Co-localization studies
-
Circuit tracing
-
Compatible with Many Imaging Techniques : Works with confocal, two-photon, light-sheet, super-resolution, and even live-cell microscopy in some cases (e.g., with calcium or voltage-sensitive dyes).
-
Activity and Functional Probes : Genetically encoded fluorescent indicators (e.g., GCaMP for calcium, SynaptoTag for synaptic activity) allow functional imaging of live neurons during activity.
-
Widely Used in Combination : Essential in CLARITY/tissue clearing, optogenetics, developmental neuroscience, and disease modeling to localize expression of genes or proteins.
Challenges/Limitation
-
Photobleaching : Fluorophores can degrade under prolonged exposure to light, reducing signal intensity during extended imaging sessions.
-
Limited Tissue Penetration : Standard fluorescence microscopy often suffers from scattering in thick tissues, limiting depth unless combined with clearing or two-photon methods.
-
Staining Quality Variability : Uneven staining, background fluorescence, or over-labeling can occur, especially in thick tissues or inconsistent antibody batches.
-
Signal Decay in Live Imaging : In live tissue, fluorescent indicators may be leaky, slow, or prone to pH sensitivity—limiting precision in functional imaging.
-
Requires Specialized Equipment : Needs high-end microscopes, proper filter sets, and often anti-fade reagents or refractive index matching media.
-
Biological Perturbation Risk : Overexpression of fluorescent proteins or long-term illumination can stress or alter cell physiology, especially in live animals.
Brainbow is a genetic technique developed to visualize individual neurons and their connections in the brain with multiple fluorescent colors. As you may easily guess the term "Brainbow" is derived from the combination of "brain" and "rainbow". it allows neurons to be labeled in a variety of distinct colors, making it easier to distinguish between them and trace their connections.
The Brainbow method involves genetically modifying neurons to express different combinations of fluorescent proteins derived from jellyfish and coral. The proteins include variants of green, red, and blue fluorescent proteins, which can be combined to generate a wide array of colors.
Appying this to a mouse brain as an example, Researchers make neurons in a mouse's brain light up in different colors. They used a genetic trick called Cre-Lox recombination to insert special genes for colorful proteins into the mouse's DNA. This way, when the neurons become active, they produce these colorful proteins and glow in various colors.
Since the color combinations are random, each neuron gets its own unique shade, making it easy to tell them apart. This colorful brain helps scientists study how neurons connect and interact in different parts of the brain.
The Brainbow method has been super helpful not only for studying mouse brains, but also for looking at other animals and even non-brain tissues.
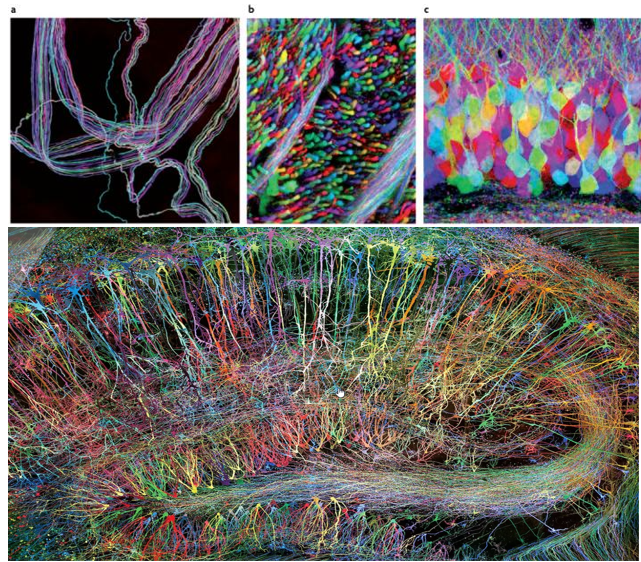
Achievement/Application
-
Multicolor Differentiation of Individual Neurons : Brainbow enables hundreds of unique color combinations by expressing different ratios of red, green, and blue fluorescent proteins—making it easy to distinguish adjacent neurons, axons, and dendrites.
-
Mapping Neuronal Circuits : Perfect for tracing complex networks of neurons, including axonal projections and dendritic arbors, especially in tightly packed brain regions like the hippocampus or cortex.
-
Single-Cell Resolution at Network Scale : Unlike traditional fluorescent staining, Brainbow allows researchers to visualize hundreds of uniquely colored neurons simultaneously, retaining single-cell resolution across broader tissue.
-
Genetically Targeted Expression : Uses Cre-lox recombination to selectively express color proteins in specific cell types or brain regions—ideal for cell-type specific tracing.
-
Compatible with CLARITY & Light-Sheet Imaging : When combined with tissue clearing and 3D microscopy, Brainbow reveals dense neural circuits in whole-brain volumes in vibrant detail.
-
Applications Beyond Neuroscience : Adapted for use in developmental biology, connectomics, and cancer research to trace lineage and interactions in other tissues.
Challenges/Limitation
-
Color Overlap and Spectral Bleed-Through : Imaging more than 3–4 fluorophores simultaneously can lead to channel bleed-through and ambiguous colors without advanced spectral unmixing.
-
Photobleaching and Signal Decay : Fluorescent proteins can degrade with exposure to light during imaging, especially in long scans of large volumes.
-
Random Expression Levels : The color combinations are stochastic, so reproducibility or precise control over which neuron expresses which color is limited.
-
Technical Complexity : Requires transgenic animals and careful breeding strategies to express Brainbow constructs, along with Cre-driver lines.
-
Limited Functional Insight : Brainbow reveals structure, not function—cannot capture activity, neurotransmitter identity, or molecular state without combining with other tools.
-
Tissue Penetration Challenges : Like other fluorescent imaging, Brainbow is limited by light scattering in thick tissue unless used with clearing methods like CLARITY.
Molecular Biology and Bioengineering as research methods in neuroscience involve studying the brain at the most fundamental biological levels—genes, proteins, and cellular mechanisms—to understand how neurons develop, communicate, adapt, and form the basis of learning, memory, and behavior. These approaches allow scientists to dissect the molecular pathways that underlie neuronal function, such as how synapses strengthen or weaken during learning or how specific genes influence neural plasticity. By manipulating genes, tracking protein expression, or engineering biological systems with precision tools like CRISPR, optogenetics, or fluorescent markers, researchers can reveal how molecular components give rise to complex neural processes. This method bridges the gap between molecular-scale events and system-level brain function, enabling not only basic discovery but also innovations in neural repair, brain-machine interfaces, and treatment strategies for neurological disorders.
This (a sea snail) would one of the most famous animal that lead to the opening of research on learning and memory at synaptic level and molecular level. This animal has very simple architecture of nervous system with much less number of neurons comparing to higher animal (this animal has only 20,000 neurons whereas a human has 100,000,000,000 neurons), but still
capable of 'learning' and 'remembering'. The size of a nerve cell is huge (in mm scale in diameter) that you can see it with naked eyes. Only small numbers of neurons (only a few hundreds) get involved in a certain behavior of the animal (Watch @11:25 of this lecture if you want further details).
Scientists was able to train this animial with a certain behavior and direct observe the changes of synaptic networks and molecular composition changes before and after the learning. You can get a pretty clear idea on how the research has been done from this short
video. If you are willing to tackle the far more details on this research, check out this lecture : Memories are Made of This. For more recent researches especially for gene expression, check out this lecture : Memories that Last: Genes Neurons and Synapses
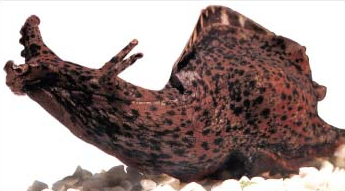
Image Souce : Wiley Online Library
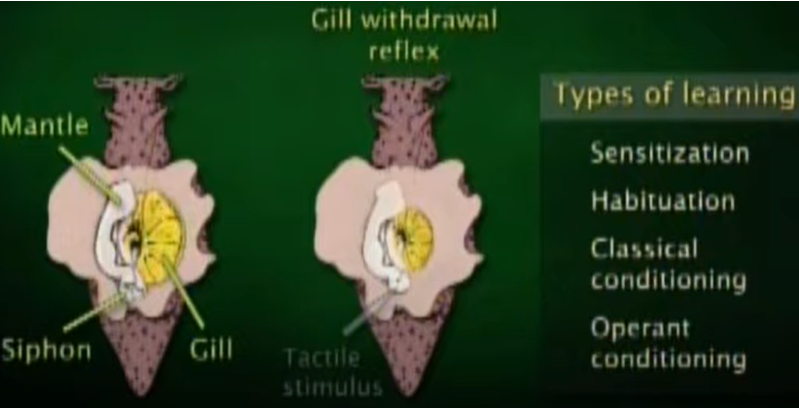
Image Source : (@13:27 of Memories are Made of This )
Common Molecular Aproaches are :
- Identification of Proteins that are involved in memory formation especially for LTP (long term potentiation)
- Investigation of the differences in terms of synaptic activity when applied with protein synthesis inhibitor
- Signal transduction mechanism for synaptic placiticity
- Identification of genes that trigger the new growth of axon termnial / dendrite
- expressing genes producing fluorescent proteins ==> this is for neuron staining (this is basis technology for Brainbow )
Achievement/Application
- Simplified Nervous System : Aplysia has only about 20,000 neurons, many of which are large enough to be seen with the naked eye—ideal for electrophysiological and molecular experiments.
- Foundation of Learning and Memory Research : Nobel laureate Eric Kandel and colleagues used Aplysia to uncover cellular and molecular mechanisms of learning, particularly:
- Sensitization
- Habituation
- Classical and Operant Conditioning
- Identifying Synaptic Plasticity Mechanisms : Studies on the gill withdrawal reflex revealed how synaptic strength changes during learning:
- Short-term memory involves presynaptic facilitation.
- Long-term memory requires gene transcription and protein synthesis.
- Link Between cAMP and Synaptic Enhancement : Aplysia studies provided the first evidence that second messengers like cAMP are critical for long-term potentiation (LTP).
- Mapping Learning to Specific Neurons : Because only a few neurons are involved in specific behaviors, researchers could directly link learning-induced changes to individual, identifiable cells.
- Basis for Molecular Labeling Tools : Discoveries in Aplysia helped identify gene expression changes, including the use of fluorescent reporter proteins—an essential basis for later tools like Brainbow and optogenetics.
- Demonstrated Behavioral Correlates : Direct connection between observed behavior changes (like increased gill withdrawal) and measurable molecular/synaptic changes, laying the foundation for molecular neuroethology.
Challenges/Limitation
- Simplified Nervous System (Strength & Limitation) : Too simple to model complex cognitive functions like emotion, attention, or executive function. It lacks the hierarchical brain architecture seen in mammals (no cortex, hippocampus, etc.).
- Lack of Genetic Manipulation Tools : Unlike model organisms like mice, zebrafish, or fruit flies, Aplysia is not genetically tractable:
- No standardized transgenic lines
- Limited gene editing capabilities
- Very few genetic markers or promoters
- Poor Translational Power to Humans : Molecular pathways (e.g., cAMP, CREB) are conserved, but human learning and memory involve many more layers of complexity, including distributed networks, attention systems, and language — none of which can be modeled in Aplysia.
- Experimental Variability : Aplysia specimens are often wild-caught, which introduces variability in:
- Age, health, environmental history
- Neuronal size and reactivity
- Makes reproducibility and standardization harder compared to lab-bred rodents or cell lines.
- Short Behavioral Repertoire : The behavioral assays (like gill withdrawal) are limited and reflexive:
- Not suitable for testing complex behaviors like navigation, decision-making, or social learning.
- Hard to generalize beyond sensorimotor conditioning.
- No Real-Time Imaging in Intact Brain : While intracellular recordings are feasible, it lacks tools for real-time in vivo imaging of the whole nervous system in awake, behaving animals.
Optogenetics is a groundbreaking research technique that combines genetics and optics to control the activity of specific neurons or other cells in living tissue using light. Thanks to this technique, researches can control the activity of specific brain cells using light. It's like a remote control for neurons!
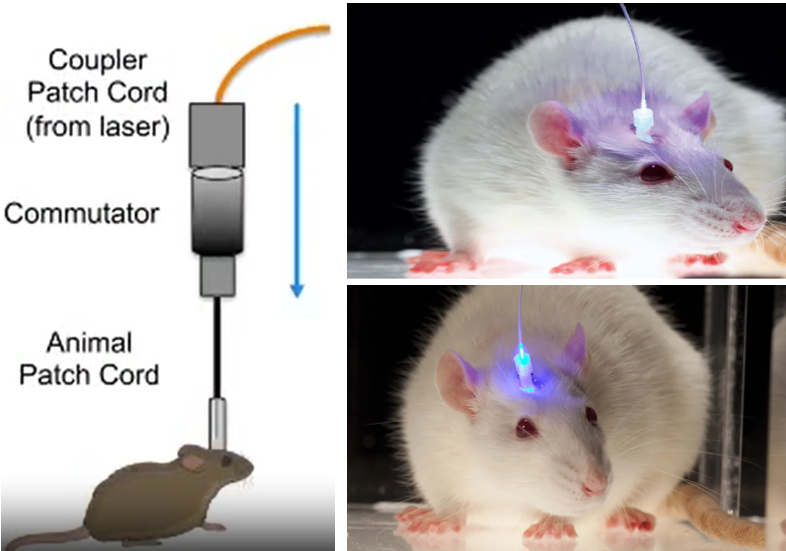
Image Sources : In vivo Optogenetic Stimulation of the Rodent Central Nervous System / Light-Based Brain Treatments May Soon Be Used On Humans
To make this work, researchers use genes from light-sensitive proteins called opsins, which are found in some algae and bacteria. They introduce these genes into the brain cells they want to control. Once the genes are inside the cells, they produce the opsins, which get incorporated into the cell membranes.
Now comes the fun part! When researchers shine light on the cells with the opsins, the opsins respond by either opening or closing ion channels in the cell membrane. This changes the electrical activity of the cells, allowing scientists to turn them "on" or "off" like a switch.
General procedure of applying this technolgy goes as follows :
- Picking the brain cells: Scientists decide which brain cells they want to work with based on the questions they're trying to answer.
- Adding the light-sensitive proteins: They put genes for special proteins called opsins into the chosen cells, usually using clever techniques like viruses or genetically modified animals.
- Producing opsins: The cells start producing these opsins and include them in their cell membranes.
- Shining the light: Scientists shine light on the cells that have opsins, usually using tiny optical fibers or LED lights. The specific color of light they use activates the opsins.
- Flipping the switch: When the opsins are activated by light, they open or close ion channels in the cells, changing their electrical activity. This lets researchers control the cells like a remote control!
Achievement/Application
- Precise Temporal Control : Light can activate or inhibit neurons within milliseconds, allowing researchers to manipulate circuits with real-time precision.
- Cell-Type Specificity : By using cell-type specific promoters or Cre-driver lines, opsins can be expressed only in specific neurons, enabling highly targeted interventions.
- Causal Manipulation of Behavior : Allows direct testing of what brain region or circuit causes a particular behavior by turning it on or off like a switch.
- Dissecting Neural Circuits : Helps determine functional connectivity between regions—e.g., stimulating axon terminals in a downstream region to trace influence back to the source.
- Therapeutic Insights : Used in models of depression, Parkinson’s disease, epilepsy, and even vision restoration by controlling damaged or inactive neurons.
- Combines with Other Techniques : Often used alongside electrophysiology, fMRI, or calcium imaging to correlate light-induced activity with neural and behavioral responses.
- Versatility Across Species : Applied in mice, rats, zebrafish, non-human primates, and even human tissues in experimental setups.
Challenges/Limitation
- Invasive Light Delivery : Requires surgical implantation of optical fibers or LEDs into the brain—making it invasive and unsuitable for chronic or human-wide application (yet).
- Limited Light Penetration : Light—especially blue light—does not penetrate deep into tissue, so only superficial or optically accessible brain regions can be targeted effectively.
- Heating and Phototoxicity : Prolonged or high-intensity light can heat the tissue or cause photodamage, potentially confounding behavioral results or damaging neurons.
- Artificial Expression of Opsins : Requires viral vector delivery or transgenic modification, which may introduce:
- Immunogenicity
- Variable expression
- Developmental artifacts
- Not a Naturalistic Stimulus : While effective, optogenetics is not mimicking natural brain inputs. The artificial spike trains it creates might differ from endogenous patterns.
- Opsin Kinetics and Trade-offs
- Different opsins (e.g., Channelrhodopsin, Halorhodopsin, ArchT) have trade-offs in:
- Light sensitivity : No single opsin is perfect for all use cases.
- Ethical and Translational Barriers : Despite huge potential, applying optogenetics to humans is still in its early stages due to ethical, technical, and safety hurdles.
Calcium imaging is a research technique that helps scientists visualize and study the activity of neurons (brain cells) by monitoring changes in calcium levels inside these cells. As you know, Calcium ions are crucial for many cellular processes, including how neurons send electrical signals. When a neuron becomes active, its calcium levels change, and calcium imaging detects these changes.
Calcium imaging has gained popularity in neuroscience because it allows researchers to study the activity of many neurons simultaneously, even in live animals. With this technology, researches can gain a better understanding of how brain cells work together to process information and control behavior.
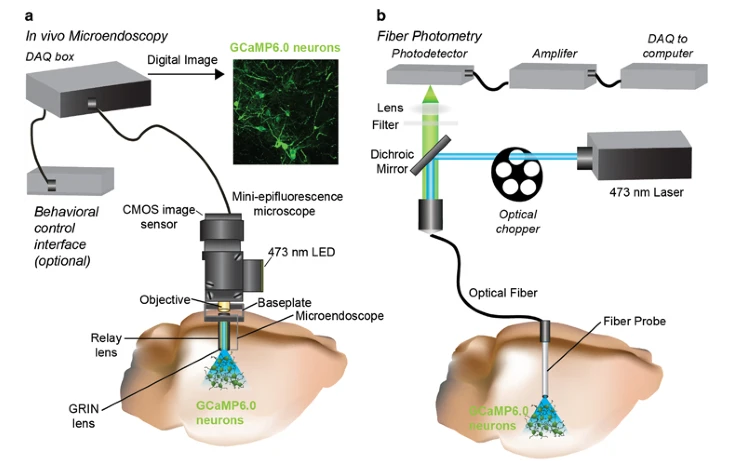
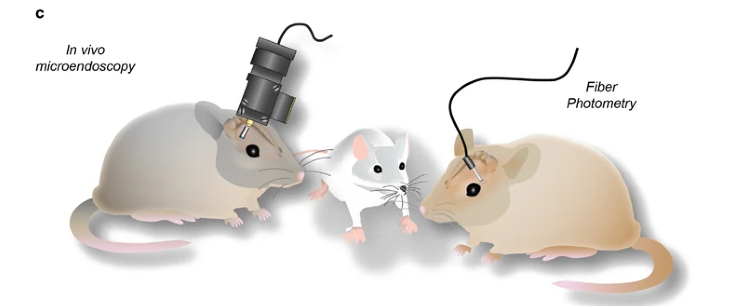
Image Source : In vivo Calcium Imaging to Illuminate Neurocircuit Activity Dynamics Underlying Naturalistic Behavior
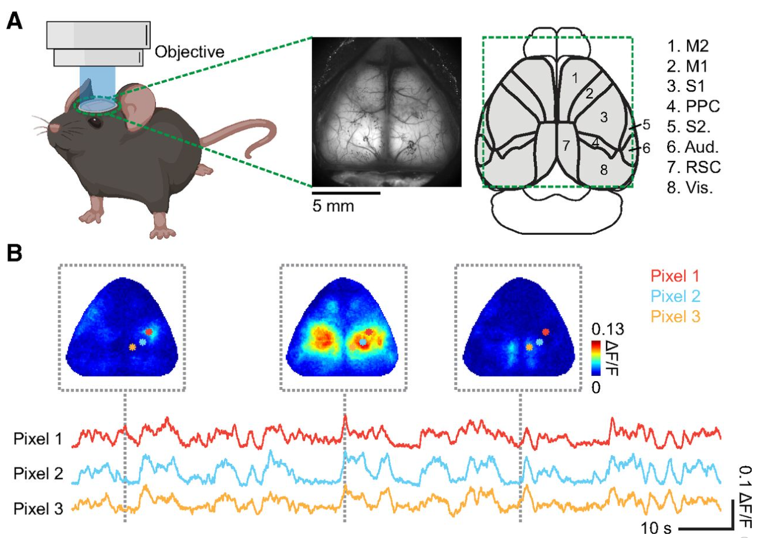
Image Source : Characterizing Cortex-Wide Dynamics with Wide-Field Calcium Imaging
To apply this techniuqe, researchers use special molecules that stick to calcium and light up when they're bound together. These molecules are called calcium indicators and they can be dyes or even glow-in-the-dark proteins made by the cells themselves.
Here's a quick and casual rundown of calcium imaging:
- Get the glow(calcium indicator) ready: Scientists add calcium indicators to the neurons they want to study. These indicators will make the cells glow when calcium levels change.
- Watch the light show: As neurons get active, the calcium indicators bind to calcium ions and start glowing. Researchers use a special microscope to watch the glowing cells and record how their brightness changes over time.
- Crunch the numbers: Scientists look at the recorded data to figure out which neurons were active and when it happened during the experiment.
Achievement/Application
- Population-Level Activity Monitoring : Allows simultaneous recording from dozens to thousands of neurons, unlike electrophysiology which is limited to fewer cells.
- Real-Time Functional Imaging : Captures neural dynamics in real-time, showing when neurons fire by detecting calcium transients (spikes in intracellular calcium).
- Cell-Type and Region Specificity : Works with genetically encoded calcium indicators (e.g., GCaMP) driven by cell-specific promoters or Cre-lines, targeting defined neuronal subtypes.
- Compatible with Behaving Animals : Techniques like fiber photometry, miniature microscopes, and wide-field imaging allow calcium activity to be tracked while animals move, explore, or interact.
- Chronic Longitudinal Studies : Enables repeated imaging of the same cells across days or weeks, ideal for learning, memory, and neuroplasticity studies.
- Integration with Other Modalities : Can be combined with:
- Optogenetics (stimulate + read activity)
- Electrophysiology
- Behavioral assays
- Deep learning pipelines for ROI extraction
Challenges/Limitation
- Indirect Measurement of Spikes : Calcium imaging is a proxy for electrical activity. Calcium indicators lag behind action potentials and have slower decay times (~100 ms – sec), so exact spike timing is blurred.
- Low Temporal Resolution Compared to Electrophysiology : Even with fast calcium sensors (like GCaMP7f), it’s still slower than millisecond precision offered by patch clamp or MEA.
- Signal Overlap and Motion Artifacts : In dense brain regions, signals from nearby cells can bleed together, and animal movement can introduce artifacts if not corrected computationally.
- Photobleaching and Phototoxicity : Long imaging sessions can degrade signal or damage tissue due to light exposure, especially with two-photon or wide-field setups.
- Limited Depth in Some Setups : One-photon techniques like miniscopes or fiber photometry sample surface layers. Two-photon microscopy is required for deep brain structures, but is bulkier and slower.
- Genetic Modification Required : Requires delivery of genetically encoded indicators (e.g., via viral vectors or transgenic lines), which may not be feasible or uniform in all systems or species.
- Complex Data Analysis : Produces high-dimensional time-series data requiring:
- Motion correction
- ROI segmentation
- Deconvolution algorithms to infer spike timing
Obviously C. elegans is not a technology or research method. It is an organism (animal). But I put this as a separate research method item because this can be utilized across such a wide spectrum.
Through research on C. elegans, scientists have gained critical insights into neural development, synaptic function, sensory perception, and the genetic and molecular bases of behavior. Moreover, studies in C. elegans have contributed to understanding the mechanisms underlying neurodegenerative diseases, such as Alzheimer's and Parkinson's disease, making it an invaluable tool in neuroscience research.
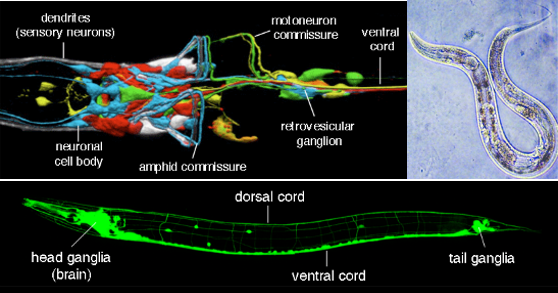
Source : C. elegans nervous system
Caenorhabditis elegans (C. elegans) plays a pivotal role in neuroscience research due to several unique and advantageous features it possesses :
- Simple Nervous System: C. elegans has a remarkably simple nervous system, consisting of only 302 neurons, which makes it an ideal model organism for studying the fundamentals of neural development, function, and behavior. This simplicity allows researchers to map all its neural connections, creating a complete wiring diagram, or "connectome," of its nervous system.
- Genetic Manipulability: The ease of genetic manipulation in C. elegans enables scientists to investigate the roles of specific genes in neural development and function. Techniques such as gene knockout, RNA interference (RNAi), and CRISPR-Cas9 gene editing allow for the precise control over gene expression, facilitating the study of genetic contributions to behavior and neurological diseases.
- Transparent Body: The transparency of C. elegans throughout its life cycle allows for direct observation of cellular processes and neural activity in living organisms. This feature is particularly valuable for in vivo studies of neuron function and neurodegenerative processes.
- Well-characterized Development and Phenotype: The developmental lineage of every cell in C. elegans is known, including its neurons. This comprehensive understanding allows researchers to study how neurons and their connections form during development and how alterations in these processes can lead to neurological disorders.
- Conserved Molecular Pathways: Many molecular pathways involved in neural function are conserved between C. elegans and higher organisms, including humans. This conservation makes it possible to extrapolate findings in C. elegans to more complex nervous systems, providing insights into human neurological diseases.
- Behavioral Studies: Despite its simplicity, C. elegans exhibits a range of behaviors, such as feeding, locomotion, and response to sensory stimuli. Studying these behaviors and the underlying neural circuits can reveal fundamental principles of nervous system organization and function.
- Rapid Life Cycle and Ease of Cultivation: The short lifespan and ease of cultivation of C. elegans allow for rapid generation turnover and large-scale genetic and pharmacological studies. This facilitates high-throughput screening for genes and compounds that affect neurological function and disease.
Experimental techniques used with Caenorhabditis elegans (C. elegans) span a wide range, leveraging its unique biological features. Here's an overview of common methods: These techniques, often used in combination, allow researchers to dissect the molecular, genetic, and neural circuit mechanisms underlying development, behavior, and disease models in C. elegans. This integrative approach has made C. elegans a powerful model organism for studying complex biological processes
- Genetic Manipulation:
- Forward Genetics: Screening for mutants with desired phenotypes followed by mapping and identifying the mutations.
- Reverse Genetics: Targeted gene disruptions using RNA interference (RNAi), CRISPR-Cas9 for gene editing, and transgenic techniques to study gene function.
- Molecular Biology Techniques:
- Fluorescent Protein Tagging: Fusion of fluorescent proteins to cellular or subcellular structures to visualize gene expression patterns, protein localization, and dynamic processes in living animals.
- Reporter Constructs: Use of reporter genes (e.g., GFP) under the control of specific promoters to study gene expression and regulation.
- Electrophysiological Recordings:
- Although more challenging in C. elegans due to its small size, patch-clamp and microelectrode techniques are adapted to record from muscles and neurons, allowing the study of electrical properties and synaptic function.
- Behavioral Assays:
- Various assays are designed to study responses to environmental stimuli (e.g., chemotaxis, thermotaxis, mechanosensation), social behavior, feeding, and locomotion. These assays help in understanding the neural circuits underlying these behaviors.
- Imaging Techniques:
- Confocal Microscopy and Multiphoton Microscopy: For high-resolution imaging of cells and tissues in live animals.
- Calcium Imaging: Utilizing calcium-sensitive fluorescent dyes or genetically encoded calcium indicators (e.g., GCaMP) to monitor neural activity in response to stimuli.
- Optogenetics:
- Manipulation of neuronal activity using light-sensitive proteins (e.g., channelrhodopsins). This technique allows precise control over the activation or inhibition of specific neurons to study their role in behavior and neural circuit function.
- Whole-Genome Sequencing and Transcriptomics:
- High-throughput sequencing techniques to study genetic variation, gene expression profiles, and regulatory networks at the whole-genome level.
- Proteomics and Metabolomics:
- Analysis of the proteome and metabolome to understand the functional consequences of genetic changes and environmental influences.
- Pharmacological Interventions:
- Use of drugs and small molecules to probe the function of neural circuits and to model the effects of pharmacological agents on behavior and physiology.
The intersection of C. elegans research with AI and neural network methodologies not only enriches our understanding of biological neural networks but also informs the development of artificial intelligence, offering a unique platform for interdisciplinary advances in both fields.
- AI/Neural Network Modeling and Simulation:
- Computational Modeling of Neural Circuits: The complete connectome of C. elegans provides an invaluable blueprint for constructing detailed computational models of neural circuits. These models enable researchers to simulate neural activity and understand how specific neural configurations contribute to behavior. AI techniques, such as machine learning algorithms, can be applied to predict outcomes of neural interactions and to optimize models based on experimental
data.
- Neural Network Research: The simplicity and fully mapped nervous system of C. elegans allow AI researchers to use it as a model to understand how neural networks can give rise to complex behaviors from simple units of computation. This research can inform the design of artificial neural networks, contributing to the development of more efficient and adaptable AI systems.
- Studying Learning and Memory: Even though C. elegans has a simple nervous system, it exhibits learning and memory behaviors. AI researchers can use these observations to inspire algorithms that mimic biological learning processes, potentially leading to AI systems capable of adaptive learning and memory storage with minimal computational resources.
- Bridging Biological and Artificial Intelligence: Insights from the study of C. elegans can inform the development of novel AI architectures that mimic biological efficiency and adaptability. Understanding how a minimal number of neurons can produce a variety of behaviors can inspire new approaches to creating lightweight AI systems for applications where computational resources are limited.
- Data Analysis and Pattern Recognition: The wealth of genetic, molecular, and behavioral data generated from C. elegans research can be analyzed using AI techniques to uncover patterns and relationships that might not be evident through traditional analysis methods. This can accelerate the discovery of key genes, neural pathways, and molecular mechanisms involved in neural function and disease.
- Personalized Medicine and Drug Discovery: AI models trained on C. elegans data can potentially predict responses to drugs and identify novel therapeutic targets for neurological diseases. By simulating the effects of drugs on C. elegans' neural circuits and comparing them with human disease models, researchers can identify promising compounds for further investigation.
- Integration of AI in Experimental Techniques:
- Automated Behavioral Analysis: AI-driven image analysis and machine vision techniques are used to automatically quantify C. elegans behaviors in various assays. This automation enables high-throughput screening and detailed behavioral phenotyping, reducing bias and increasing the efficiency of research.
- Enhanced Imaging Analysis: AI algorithms are applied to enhance the analysis of imaging data from C. elegans, such as identifying specific neurons in fluorescent microscopy images or tracking neural activity over time. These algorithms can deal with the complexity of dynamic biological systems, providing deeper insights into neural function and development.
YouTube
- Brain Lesion
- Electro Recording
- MRI/fMRI
- Volume Electron Microscope
- CLARITY/Tissue Clearing
- Micro / Molecular Biology
- Staining
- Real Time Monitoring of Neuronal activity
- Two Photon Microsocopy
- Molecular Biology/Bio Engineering
- Human Connectome Project
- Neurons on a chip
- Generics
- Optogenetics
- Calcium Imaging
Reference
|
|